2. Maintaining a balance

What will I be able to do at the end of this chapter?
- Describe the structure of the human body in terms of six levels of organisation
- Describe the structure and function of the principal components of the cell
- Identify the main tissue types and discuss their roles in the human body
- Define homeostasis and explain its importance to normal human functioning.
This chapter begins with an explanation of the hierarchical organisation of the human body, from the simplest (cell) to the most complex (organ system) level. It describes the basic structure of the cell and the function of principle cell components, and then goes on to introduce the basic tissue types of the human body. A discussion on the concept of homeostasis and how the body works to maintain stable conditions for itself concludes the chapter.
2.1 | How is the human body organised?
Before you begin to study the different structures and functions of the human body, it is helpful to consider its basic architecture; that is, how its smallest parts are assembled into larger structures.
Life processes of the human body are maintained at several levels of structural organisation. These include the chemical, cellular, tissue, organ, organ system, and the organism level. Higher levels of organisation are built from lower levels. Therefore, molecules combine to form cells, cells combine to form tissues, tissues combine to form organs, organs combine to form organ systems, and organ systems combine to form organisms.
It is convenient therefore to consider the structures of the body in terms of fundamental levels of organisation that increase in complexity: atoms, molecules, cells, tissues, organs, organ systems.
Levels of organisation

The organisation of the body is often discussed in terms of six distinct levels of increasing complexity, from the smallest chemical building blocks to the full human organism (Figure 2.2).
To study the chemical level of organisation, scientists consider the simplest building blocks of matter: subatomic particles, atoms and molecules. All matter in the universe is composed of one or more unique pure substances called elements, familiar examples of which are hydrogen, oxygen, carbon, nitrogen, calcium, and iron. The smallest unit of any of these pure substances (elements) is an atom. Atoms are made up of subatomic particles such as the proton, electron and neutron. Two or more atoms combine to form a molecule, such as water, proteins, fats and sugars found in living things. The large molecules necessary for life are called biological macromolecules (biomolecules). Biomolecules are molecules that are the chemical building blocks of all body structures.
Cells contain four major macromolecules, including nucleic acids (DNA/RNA), proteins, lipids, and carbohydrates. Each has distinct structural and biochemical properties that lends to its individual functions within the cell. Collectively, the macromolecules create the molecular players, structures, and organelles that perform tasks within the cell. Table 2.1 outlines some of these features for you just to provide an overview of their structure and function; however, these macromolecules will be explored in greater detail in later chapters.
Macromolecule | Building block (repeating unit) | Major functions |
Protein | Amino acids | Fundamental to structure and function of the cell |
Nucleic acid (DNA) | Nucleotides | Repository of hereditary information; essential for protein synthesis |
Carbohydrate | Monosaccharides (e.g. glucose) | Storage form of energy to meet short term demands |
Lipid (fat) | Fatty acids | Storage form of energy to meet longer term demand; structural components of membranes |
The next level of organisation is the cell itself. A cell is the smallest independently functioning unit of a living organism. Even bacteria, which are extremely small, independently living organisms, have a cellular structure. Each bacterium is a single cell. All living structures of human anatomy contain cells, and almost all functions of human physiology are performed in cells or are initiated by cells.
A human cell typically consists of flexible membranes that enclose cytoplasm, a water-based cellular fluid, together with a variety of tiny functioning units called organelles (Figure 2.3).

A tissue is a group of many similar cells (though sometimes composed of a few related types) that work together to perform a specific function. An organ is an anatomically distinct structure of the body composed of two or more tissue types. Each organ performs one or more specific physiological functions. As we saw in Chapter 1: Talking about the human body, an organ system is a group of organs that work together to perform major functions or meet physiological needs of the body.
2.2 | How does the structure and function of the cell affect an organism?
Ever wonder how paracetamol works to alleviate your headache? Do you know why the caffeine in your coffee helps to kick-start your day? What causes mucosal build up in the lungs of individuals with cystic fibrosis? What is the best way to treat a patient diagnosed with diabetes? The answer to all of these questions begins with an understanding of cell biology, in other words the structure and function of a cell.
Close your eyes and picture a brick wall. What is the basic building block of that wall? It is a single brick, of course. Like a brick wall, your body is composed of basic building blocks, and the building blocks of your body are cells. The cell is the smallest functional unit of a living organism.
While some organisms are comprised of just a single cell (prokaryotes), humans (eukaryotes) are much more complex and contain about 30 trillion cells that function together to provide you with legs to walk to class, a digestive system to refuel after a long night of studying, and brain power to learn subjects like anatomy and physiology.
Before we can tackle these big physiological questions about your body, it is important that we take a closer look at the foundation of each organ system: the eukaryotic cell. Cells were first recognised about 300 years ago by Robert Hooke, an English scientist who noted the compartmentalisation of a cork (the outer bark of many trees) slice under the magnification of a microscope. The sturdy cell walls of the plant specimen he observed were published in Micrographia in 1665 (Figure 2.4). It was in this publication that Hooke coined the term “cell” from the Latin word cellula (small chamber). Shortly thereafter, Antonie van Leeuwenhoek, a Dutch microscopist, began visualising live microorganisms such as bacteria which he called “animalcules”) along with specialised cell types in multicellular organisms, including muscle fibres, red blood cells, and sperm. It was not until another 200 years passed, however, that scientists began to understand the function of the cell. As microscopes became more advanced, it became easier to study the behaviours within these compartments, and by the mid-1800s, the pillars of the cell theory emerged (YouTube video [6:11]).

Your body has many kinds of cells, each specialised for a specific purpose [Core Concept: structure and function]. Just as a home is made from a variety of building materials, the human body is constructed from many cell types. For example, epithelial cells protect the surface of the body and cover the organs and body cavities within. Bone cells help to support and protect the body. Cells of the immune system fight invading bacteria. Additionally, red blood cells carry oxygen throughout the body. Each of these cell types plays a vital role during the growth, development, and day-to-day maintenance of the body. In spite of their enormous variety, however, all cells share certain fundamental characteristics.
How do we know what cells look like?
Microscopy
Cells vary in size. With few exceptions, individual cells are too small to be seen with the naked eye, so scientists use microscopes to study them. A microscope is an instrument that magnifies an object. Most images of cells are taken with a microscope and are called micrographs.
Light microscopes
To give you a sense of the size of a cell, a typical human red blood cell is about eight millionths of a meter or eight micrometres (abbreviated as µm) in diameter; the head of a pin is about two thousandths of a metre in diameter. That means that approximately 250 red blood cells could fit on the head of a pin.
Use the slide beneath the image in this interactive demonstration of cell size and scale.
Light microscopes use two sets of lenses to magnify the image. Due to the manner in which light travels through the lenses, this system of lenses produces an inverted image. Light microscopes are advantageous for viewing living organisms, but since individual cells are generally transparent, their components are not distinguishable unless they are coloured with special stains. Staining, however, usually kills the cells.

Compound light microscopes (Figure 2.5a) commonly used in the undergraduate college laboratory magnify up to approximately 400 times. Magnification is usually increased to 1,000 times for the study of smaller cells, like most prokaryotic cells (e.g. bacteria) but this usually requires a special oil immersion lens. The light comes from below the specimen and must pass through the specimen and then be focused onto the eye of the observer. This means that the specimen sample must be really thin, or translucent to allow the light through.

A second type of light microscope used in laboratories is the dissecting microscope (Figure 2.5b). These microscopes have a lower magnification (20 to 80 times the object size) than light microscopes and can provide a three-dimensional view of the specimen. Thick objects can be examined with many components in focus at the same time. These microscopes are designed to give a magnified and clear view of tissue structure as well as the anatomy of the whole organism. Dissecting microscopes also have optics that correct the image so that it appears as if being seen by the naked eye and not as an inverted image. The light illuminating a sample under a dissecting microscope typically comes from above the sample but may also be directed from below.
Electron microscopes
In contrast to light microscopes, electron microscopes use a beam of electrons instead of a beam of light. There are two types of electron microscopes, the scanning electron microscope (SEM) and the transmission electron microscope (TEM); the former provides details of a specimen’s surface whereas the latter focuses on the internal composition of the specimen. Some electron microscopes can magnify a specimen up to two million times.
Note that preparation of a specimen for viewing under an electron microscope will kill it; therefore, live cells cannot be viewed using this type of microscopy. In addition, the electron beam moves best in a vacuum, making it impossible to view living materials.

Career Connection: Cytotechnologist
Have you ever heard of a medical test called a Pap smear? In this test, a doctor takes a small sample of cells from the uterine cervix of a patient and sends it to a medical lab where a cytotechnologist stains the cells and examines them for any changes that could indicate cervical cancer or a microbial infection (Figure 2.7).
Cytotechnologists (cyto = cell) are professionals who study cells through microscopic examinations and other laboratory tests. They are trained to determine which cellular changes are within normal limits or are abnormal. Their focus is not limited to cervical cells; they study cellular specimens that come from all organs. When they notice abnormalities, they consult a pathologist, who is a medical doctor who can make a clinical diagnosis.
Cytotechnologists play vital roles in saving people’s lives. When abnormalities are discovered early, a patient’s treatment can begin sooner, which usually increases the chances of the treatment being successful.

What are the parts of a cell?
As mentioned earlier, there are many types of cells, and all are grouped into one of two broad categories: prokaryotic and eukaryotic. In this subject we are more interested in eukaryotic cells, which are the type of cells found in humans (and also in other animals and plants). Prokaryotic cells are mostly found in bacteria – which you will cover in more detail in your microbiology subjects. All cells share four common components:
1) a plasma membrane, or cell membrane, an outer covering that separates the cell’s interior from its surrounding environment;
2) cytoplasm, consisting of a jelly-like region within the cell in which other cellular components are found;
3) DNA, the genetic material of the cell; and
4) ribosomes, particles that synthesise proteins.
How are prokaryotic and eukaryotic cells different?
Prokaryotic cells
However, prokaryotes differ from eukaryotic cells in several ways. A prokaryotic cell is a simple, single-celled (unicellular) organism that lacks a nucleus, or any other membrane-bound organelle. We will shortly come to see that this is significantly different in eukaryotes. Prokaryotic DNA is found in the central part of the cell: a darkened region called the nucleoid (Figure 2.8).

Unlike eukaryotes, bacteria have a cell wall in addition to the cell membrane (Figure 2.8) which acts as an extra layer of protection, helps the cell maintain its shape, and prevents dehydration. The capsule enables the cell to attach to surfaces in its environment. Some prokaryotes have flagella, pili, or fimbriae. Flagella are used in nature, for locomotion, while most pili are used to exchange genetic material during a type of reproduction called conjugation.
The relationship between structure and function is apparent at all levels, including at the level of the cell, and this will become clear as we explore eukaryotic cells.
Eukaryotic cells

A eukaryotic cell (Figure 2.9) is a cell that has a membrane-bound nucleus and other membrane-bound compartments or sacs, called organelles, which have specialised functions. The word eukaryotic means “true kernel” or “true nucleus”, alluding to the presence of the membrane-bound nucleus in these cells. The word organelle means “little organ,” organelles have specialised cellular functions, just as the organs of your body have specialised functions. At this point, it should be clear that eukaryotic cells have a more complex structure than prokaryotic cells.
The cell membrane
The cell membrane (also called the plasma membrane) is the physical boundary between the intracellular space (the inside of the cell) and the extracellular environment (the outside of the cell). It acts almost like the “skin” of the cell, controlling the movement of substances in and out of cells. The cell membrane is semi-permeable, allowing only select ions and organic molecules to enter and/or leave the cell. Therefore, it is selectively permeable. The cell membrane consists of two layers of phospholipids (also referred to as the lipid bilayer) with embedded proteins that have numerous functions (Figure 2.10). The structure and function of the cell membrane will be discussed in greater detail in Chapter 5: Membrane transport.

The cytoplasm
The cytoplasm comprises the contents of a cell between the plasma membrane and the nuclear envelope (a structure to be discussed shortly). It is made up of organelles suspended in the gel-like cytosol (Figure 2.9), the cytoskeleton, and various chemicals. Even though the cytoplasm consists of 70 to 80 percent water, it has a semi-solid consistency, which comes from the proteins within it. However, proteins are not the only organic molecules found in the cytoplasm; glucose and other monosaccharides, polysaccharides, amino acids, nucleic acids, fatty acids are also found there. In addition, ions of sodium, potassium, calcium, and many other elements are dissolved in the cytoplasm. Many chemical reactions, essential for the functioning of the cell, take place in the cytoplasm.
The cytoskeleton
If you were to remove all the organelles from a cell, would the plasma membrane and the cytoplasm be the only components left? No. Within the cytoplasm, there would still be ions and organic molecules, plus a network of protein fibres that helps to maintain the shape of the cell secures certain organelles in specific positions, allows cytoplasm and vesicles to move within the cell, and enables unicellular organisms to move independently. Collectively, this network of protein fibres is known as the cytoskeleton. There are three types of fibres within the cytoskeleton: microfilaments, also known as actin filaments, intermediate filaments, and microtubules (Figure 2.11).

The centrosome (Figure 2.9) is a region near the nucleus of animal cells that functions as a microtubule-organising centre. It contains a pair of centrioles, two structures that lie perpendicular to each other. Each centriole is a cylinder of nine triplets of microtubules. The centrioles are important in pulling the duplicated chromosomes to opposite ends when a cell divides.
Flagella and cilia
Flagella (singular = flagellum) are long, hair-like structures that extend from the plasma membrane, and are used to move an entire cell, (for example, sperm). When present, the cell has just one flagellum or a few flagella; when cilia (singular = cilium) are present, however, they are many in number and extend along the entire surface of the plasma membrane. They are short, hair-like structures that are used to move substances along the outer surface of the cell (for example, the cilia of cells lining the fallopian tubes that move the ovum toward the uterus, or cilia lining the cells of the respiratory tract that move particulate matter that mucus has trapped, toward the throat.
The endomembrane system
The endomembrane system (endo = within) is a group of membranes and organelles (Figure 2.12) in eukaryotic cells that work together to modify, package, and transport lipids and proteins.
Although not technically within the cell, the plasma membrane is included in the endomembrane system because, as you will see, it interacts with the other endomembranous organelles.
The nucleus
Typically, the nucleus is the most prominent organelle in a cell (Figure 2.9). The nucleus (plural = nuclei) houses the cell’s DNA in the form of chromatin and directs the synthesis of ribosomes and proteins. Let us look at it in more detail (Figure 2.12).

The nuclear envelope is a double-membrane structure that constitutes the outermost portion of the nucleus. Both the inner and outer membranes of the nuclear envelope are phospholipid bilayers.
The nuclear envelope is punctuated with pores that control the passage of ions, molecules, and RNA between the nucleoplasm and the cytoplasm. Like DNA, RNA is built from nucleic acids. RNA is instrumental in converting the information stored in DNA into proteins, which are in turn essential for the structure and function of cells. The process of how information is carried from DNA to protein is discussed in greater detail in Chapter 6: Coding life.
To understand chromatin, it is helpful to first consider chromosomes. Chromosomes are structures within the nucleus that are made up of DNA, the hereditary material, and proteins. This combination of DNA and proteins is called chromatin. In eukaryotes, chromosomes are linear structures. Every species has a specific number of chromosomes in the nucleus of its body cells. For example, in humans, the chromosome number is 46, whereas in fruit flies, the chromosome number is eight.
Chromosomes are only visible and distinguishable from one another when the cell is getting ready to divide. When the cell is in the growth and maintenance phases of its life cycle, the chromosomes resemble an unwound, jumbled bunch of threads.
We already know that the nucleus directs the synthesis of ribosomes, but how does it do this? Some chromosomes have sections of DNA that encode ribosomal RNA. A darkly staining area within the nucleus, called the nucleolus aggregates the ribosomal RNA with associated proteins to assemble the ribosomal subunits that are then transported through the nuclear pores into the cytoplasm; this process will be discussed in more detail again in Chapter 6: Coding Life.
The endoplasmic reticulum
The endoplasmic reticulum (ER) (Figure 2.9) is a series of interconnected membranous tubules that collectively modify proteins and synthesise lipids. However, these two functions are performed in separate areas of the endoplasmic reticulum: the rough endoplasmic reticulum (RER) and the smooth endoplasmic reticulum (SER), respectively.
The hollow portion of the ER tubules is called the lumen. The membrane of the ER, which is a phospholipid bilayer embedded with proteins, is continuous with the nuclear envelope.
The rough endoplasmic reticulum is so named because the ribosomes attached to its cytoplasmic surface give it a studded appearance when viewed through an electron microscope.
The ribosomes synthesise proteins while attached to the ER, resulting in transfer of their newly synthesised proteins into the lumen of the RER where they undergo modifications such as folding or addition of sugars. The RER also makes phospholipids for cell membranes. If the phospholipids or modified proteins are not destined to stay in the RER, they will be packaged within vesicles and transported from the RER by budding from the membrane (Figure 2.15). Since the RER is engaged in modifying proteins that will be secreted from the cell, it is abundant in cells that secrete proteins, such as in the liver.
The smooth endoplasmic reticulum is continuous with the RER but has few or no ribosomes on its cytoplasmic surface (see Figures 2.9 and 2.15). The SER’s functions include synthesis of carbohydrates, lipids (including phospholipids), and steroid hormones, detoxification of medications and poisons, alcohol metabolism, and storage of calcium ions.
The Golgi apparatus
We have already mentioned that vesicles can bud from the ER, but where do the vesicles go? Before reaching their final destination, the lipids or proteins within the transport vesicles need to be sorted, packaged, and tagged so that they wind up in the right place. The sorting, tagging, packaging, and distribution of lipids and proteins take place in the Golgi apparatus (also called the Golgi body), a series of flattened membranous sacs (Figures 2.9, 2.13 and 2.15).

The Golgi apparatus has a receiving face (cis) near the endoplasmic reticulum and a releasing face (trans) on the side away from the ER, toward the cell membrane. The transport vesicles that form from the ER travel to the receiving face, fuse with it, and empty their contents into the lumen of the Golgi apparatus. As the proteins and lipids travel through the Golgi, they undergo further modifications. The most frequent modification is the addition of short chains of sugar molecules. The newly modified proteins and lipids are then tagged with small molecular groups to enable them to be routed to their proper destinations.
Finally, the modified and tagged proteins are packaged into vesicles that bud from the opposite face (trans) of the Golgi (Figure 2.13). While some of these vesicles, transport vesicles, deposit their contents into other parts of the cell where they will be used, others, secretory vesicles, fuse with the plasma membrane and release their contents outside the cell.
The amount of Golgi in different cell types again illustrates that form follows function within cells. Cells that engage in a great deal of secretory activity (such as cells of the salivary glands that secrete digestive enzymes or cells of the immune system that secrete antibodies) have an abundant number of Golgi.
Lysosomes
In animal cells, the lysosomes are the cell’s garbage disposal. Digestive enzymes within the lysosomes aid the breakdown of proteins, polysaccharides, lipids, nucleic acids, and even worn-out organelles. These enzymes are active at a much lower pH (more acidic) than those located in the cytoplasm. Many reactions that take place in the cytoplasm could not occur at a low pH, thus the advantage of compartmentalising the eukaryotic cell into organelles is apparent.
Lysosomes also use their enzymes to destroy disease-causing organisms that might enter the cell. A good example of this occurs in a group of white blood cells called macrophages, which are part of your body’s immune system. In a process known as phagocytosis (Figure 2.14), a section of the plasma membrane of the macrophage invaginates (folds in) and engulfs a pathogen. The invaginated section, with the pathogen inside, then pinches itself off from the plasma membrane and becomes a vesicle. The vesicle fuses with a lysosome. The lysosome’s enzymes then destroy the pathogen.

Vesicles and vacuoles
Vesicles and vacuoles are membrane-bound sacs that function in storage and transport. Vacuoles are somewhat larger than vesicles, and the membrane of a vacuole does not fuse with the membranes of other cellular components. Vesicles can fuse with other membranes within the cell system.

Ribosomes
Ribosomes are the cellular structures responsible for protein synthesis. They themselves are made up of RNA and protein. Electron microscopy has shown that ribosomes consist of large and small subunits. Ribosomes are enzyme complexes that are responsible for protein synthesis.
Because protein synthesis is essential for all cells, ribosomes are found in practically every cell. They are particularly abundant in immature red blood cells for the synthesis of haemoglobin, which functions in the transport of oxygen throughout the body.
Mitochondria
Mitochondria (singular = mitochondrion) are often called the powerhouses or energy factories of a cell because they are responsible for making adenosine triphosphate (ATP), the cell’s main energy-carrying molecule. The formation of ATP from the breakdown of glucose is known as cellular respiration. Mitochondria are oval-shaped, double-membrane organelles (Figure 2.16) that have their own ribosomes and DNA. Each membrane is a phospholipid bilayer embedded with proteins. The inner layer has folds called cristae, which increase the surface area of the inner membrane. The area surrounded by the folds is called the mitochondrial matrix. The cristae and the matrix have different roles in cellular respiration.
In keeping with our theme of form following function, it is important to point out that muscle cells have a very high concentration of mitochondria because muscle cells need a lot of energy to contract.
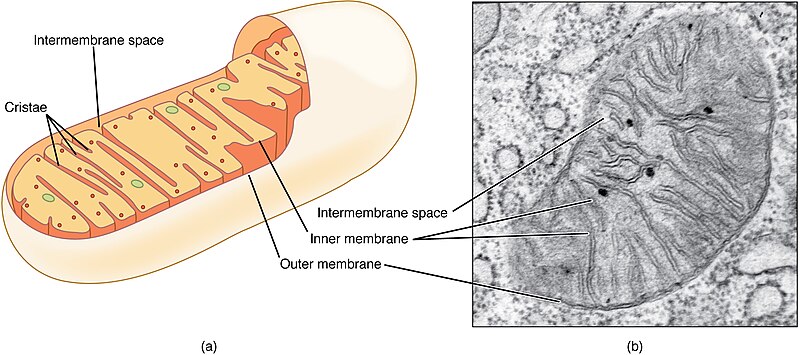
Crossword puzzle
2.3 | What are the 4 tissues of the human body?
The next level of organisation is the tissue level. A tissue is a group of connected cells that have a similar function within an organism. There are four basic types of tissues in the bodies of all animals including the human body. These make up all the organs, structures, and other contents of the body. The four basic types of tissues are muscle, epithelial, nervous, and connective. Figure 2.17 shows an example of each tissue type.




Muscle tissue
Encompasses not only the muscles, such as those in our legs or fingers, which we actively control, but also the tissue that forms most of our internal organs. There are three types of muscle tissue: skeletal, cardiac, and smooth. Skeletal muscle tissue forms what we think of as our muscles; it is attached to our bones by our tendons and can be relaxed or contracted voluntarily. Similar in structure to skeletal muscle, cardiac muscle is found exclusively in the walls of the heart. The major difference, however, is that cardiac muscle is involuntary and cannot be actively controlled. Similarly, smooth muscle, which forms the muscle layers in internal organs such as the digestive tract and bladder, is an involuntary tissue. Smooth muscle tissue controls slow involuntary movements such as stomach wall contractions, and the contractions of arteries to regulate blood flow.
Epithelial tissue
Epithelial tissue is made up of a layer or layers of tightly packed cells that line the surfaces of the body. The largest example of epithelial tissue (also the largest organ in the human body) is the skin. Mammalian skin consists of stratified epithelium, which has several layers of cells. The outermost layers of cells, called squamous cells, are flat plate-like cells, while the deeper layers are roughly cube shaped and called cuboidal cells. Epithelial tissue has multiple functions, but it serves primarily to protect, absorb, and secrete. Our skin organ covers our entire body and protects underlying tissues from bacteria, chemicals, and other injury. Epithelial cells also line the oviduct as well as the small intestine where they absorb nutrients, and similar cells in the glands secrete enzymes and hormones.
Nervous tissue
Nervous tissue is made up of the neurons and glia which are specialised cells that form the nervous system, including the brain and spinal cord. Neurons are especially responsive to stimuli, allowing nervous tissue to transmit stimuli from the brain to the body extremely rapidly.
Connective tissue
Connects, supports, or separates other tissues and organs. Connective tissue proper, a form of connective tissue, can be either loose or dense. Adipose tissue, or fat, is loose connective tissue, while tendons and ligaments, composed of collagen, are examples of dense connective tissue. Other forms of connective tissue include blood (fluid connective tissue) and cartilage and bone (both forms of supporting connective tissue).
Disorders of the mitochondria
Over the past 10 years, mitochondrial defects have been implicated in a variety of degenerative diseases, aging, and cancer. However, the pathophysiology of mitochondrial diseases remains perplexing. One of the first indications that mitochondria may play a role in pathogenesis was reported nearly 40 years ago. A patient with hyper-metabolism had skeletal muscle that contained large numbers of abnormal mitochondria, a condition now known as mitochondrial myopathy. Patients with mitochondrial myopathy suffer from muscle weakness and exercise intolerance, stroke like episodes, dementia, blindness, deafness, heart failure, arrhythmias, and movement disorders. Mitochondrial myopathies encompass an extraordinary assemblage of clinical problems, commonly involving tissues that have high-energy requirements such as heart, muscle, and the renal and endocrine systems.
2.4 | What is homeostasis?
To function properly, cells require appropriate conditions such as proper temperature, pH, and concentrations of diverse chemicals. These conditions may, however, change from one moment to the next. Organisms are able to maintain internal conditions within a narrow range almost constantly, despite environmental changes, through a process called homeostasis or steady state— the ability of an organism to maintain constant internal conditions. For example, many organisms regulate their body temperature in a process known as thermoregulation. Organisms that live in cold climates, such as the polar bear (Figure 2.18), have body structures that help them withstand low temperatures and conserve body heat. In hot climates, organisms have methods (such as perspiration in humans or panting in dogs) that help them to shed excess body heat.

Eleven distinct body systems exist in the human body. These systems can function alone, however, they need to work together to make a living organism.
Each body system contributes to the homeostasis of other systems and of the entire organism. No system of the body works in isolation, and the well-being of the person depends upon the well-being of all the interacting body systems. A disruption within one system generally has consequences for several other body systems.
Maintaining homeostasis requires that the body continuously monitor its internal conditions. Each physiological parameter or condition (such as body temperature, blood pressure, levels of certain nutrients) has a particular set point. A set point is the physiological value around which the normal range fluctuates. A normal range is the restricted set of values that is optimally healthful and stable. For example, the set point for normal human body temperature is approximately 37°C (98.6°F). Physiological parameters, such as body temperature and blood pressure, tend to fluctuate within a normal range a few degrees above and below that point. Control centres in the brain and other parts of the body monitor and react to deviations from homeostasis using negative feedback. Negative feedback is a mechanism that reverses a deviation from the set point. Therefore, negative feedback maintains body parameters within their normal range. The maintenance of homeostasis by negative feedback goes on throughout the body at all times, and an understanding of negative feedback is thus fundamental to an understanding of human physiology.
Negative feedback
A negative feedback system has three basic components (Figure 2.19). A sensor, also referred to as a receptor, is a component of a feedback system that monitors a physiological value. This value is reported to the control centre. The control centre is the component in a feedback system that compares the value to the normal range. If the value deviates too much from the set point, then the control centre activates an effector. An effector is the component in a feedback system that causes a change to reverse the situation and return the value to the normal range.

Example: Negative feedback loop for regulation of body temperature
One of the many parameters that the body must maintain within a normal range is body temperature. Body temperature must be kept within the normal range so that the chemical processes undertaken by the cells can proceed, and so that blood flow through the body is sufficient to supply nutrients to, and remove wastes from, cells.
The body can be divided into two compartments for the measurement of temperature: the skin, which fluctuates more widely along with temperatures in the external environment, and the core, which tends to be more stable . Body temperature measured externally in the armpit (axillary) can fluctuate between 35.01°C and 36.93°C. Rectal measurement is the most accurate way to measure core body temperature and this measurement can vary between 36.32°C and 37.76°C. If body temperature increases or decreases to temperatures outside of this normal range, homeostatic mechanisms will be activated to reverse the change in temperature and bring core temperature back to close to 36.8°C. This is an example of a negative feedback loop.
Body temperature is monitored by nerve cells called thermoreceptors throughout the body, including in the skin and brain. Nerve signals are sent from these receptors to the control centre, where they are integrated, and a response is formulated. For thermoregulation, the control centre is located in the hypothalamus of the brain. Chemical and nervous signals are sent from the control centre to the appropriate effectors that will act to cause a response that will reverse the temperature change.
For example, if body temperature increases (stimulus), this is detected by the thermoreceptors (receptor), which send a signal to the hypothalamus (control centre). The sweat gland and blood vessels are two of the key effectors for reducing body temperature. Blood vessels (effector) will dilate (response) and sweat glands (effector) will release sweat (response). The effect of these actions is: the increase in the diameter of the blood vessels allows more blood to come from the core of the body to the surface, where sweat evaporates causing a cooling effect that cools the blood. Once the body temperature returns to normal, negative feedback will cause the response to end (see Figure 2.20). This sequence of stimulus-receptor-signal-response is used throughout the body to maintain homeostasis.
If, on the other hand, body temperature decreases (stimulus), the pathway through the negative feedback loop is similar: the stimulus is detected by the thermoreceptors, a signal is passed to the hypothalamus (control centre). However, this time the effectors and responses are slightly different, the blood vessels (effector) are still involved, but they will constrict (response), reducing the flow of blood to the surface of the body. In addition, the muscles (effector) of the hairs on the arms and legs contract causing the hairs to stand up (response) and trap a layer of air which can be warmed and provide a small amount of insulation. The larger skeletal muscles (effector) may also begin to contract and cause shivering (response), which can generate heat through metabolic pathways.

If the body’s homeostatic mechanisms fail, then there is a risk of death or illness. For example, in very hot environments or where people are engaged in highly energetic activities, there can be a risk of hyperthermia such as heat exhaustion and heat stroke. The definition of hyperthermia is a core body temperature greater than 40°C. Symptoms of hyperthermia include sudden dizziness, confusion, fainting, cramps, nausea. If left untreated, hyperthermia can lead to breakdown of muscle tissue known as rhabdomyolysis [side: myo = muscle; lysis = breakdown] and kidney failure. The most important treatment for hyperthermia is to decrease the body temperature and this can be done with cooling blankets, ice packs and cold showers .
Equally, in very cold environments there is a risk of hypothermia. Hypothermia is defined as a core body temperature below 35°C and becomes life-threatening if core body temperature drops below 32°C . Symptoms of hypothermia may include uncontrollable shivering (although this may stop as hypothermia progresses), confusion and drowsiness, slowing of breathing and heart rate and coma. If a person is hypothermic, the most important treatment is to warm the body, particularly in “core” areas such as chest, neck, head and groin (as blood flow will be decreased to peripheral areas due to vasoconstriction).
Positive feedback loops
Some processes in the body are regulated by positive feedback. Positive feedback intensifies a change in the body’s physiological condition rather than reversing it. A deviation from the normal range results in more change, and the system moves farther away from the normal range. Positive feedback in the body is normal only when there is a definite end point. Childbirth and the body’s response to blood loss are two examples of positive feedback loops that are activated only when needed.
Childbirth at full term is an example of a situation in which the maintenance of the existing body state is not desired. Enormous changes in the mother’s body are required to expel the baby at the end of pregnancy. The events of childbirth, once begun, must progress rapidly to a conclusion or the lives of the mother and the baby are at risk. The extreme muscular work of labour and delivery are the result of a positive feedback system (Figure 2.21).

The first contractions of labour (the stimulus) push the baby toward the cervix (the lowest part of the uterus). The cervix contains stretch-sensitive nerve cells (the sensors) that monitor the degree of stretching. These nerve cells send messages to the brain (the hypothalamus is the control centre), which in turn causes the pituitary gland at the base of the brain to release the hormone into the bloodstream. Oxytocin causes stronger contractions of the smooth muscles (the effectors) of the uterus, pushing the baby further down the birth canal. This causes even greater stretching of the cervix. The cycle of stretching, oxytocin release, and increasingly more forceful contractions stops only when the baby is born. At this point, the stretching of the cervix halts, stopping the release of oxytocin into the bloodstream.
A second example of positive feedback centres on reversing extreme damage to the body. Following a penetrating wound, the most immediate threat is excessive blood loss. Less blood circulating means reduced blood pressure and reduced perfusion (penetration of blood) to the brain and other vital organs. If perfusion is severely reduced, vital organs will shut down and the person will die. The body responds to this potential catastrophe by releasing substances in the injured blood vessel wall that begin the process of blood clotting. As each step of clotting occurs, it stimulates the release of more clotting substances. This accelerates the processes of clotting and sealing off the damaged area. Clotting is contained in a local area based on the tightly controlled availability of clotting proteins. This is an adaptive, life-saving cascade of events.
cell biology the study of living organisms at the cellular levelcell theory scientific theory that describes the properties of a cell
cell membrane membrane surrounding all animal cells, composed of a phospholipid bilayer interspersed with various molecules; also known as plasma membrane
control centre compares values to their normal range; deviations cause the activation of an effector
cytoplasm internal material between the cell membrane and nucleus of a cell, mainly consisting of a water-based fluid called cytosol, within which are all the other organelles and cellular solute and suspended materials
cytoskeleton a network of protein fibres that helps to maintain the shape of the cell, secures certain organelles in specific positions, allows cytoplasm and vesicles to move within the cell, and enables unicellular organisms to move independently.
DNA is the material that carries all the information about how a living thing will look and function
development changes an organism goes through during its life
differentiation process by which unspecialised cells become specialised in structure and function
endomembrane system the group of organelles and membranes in eukaryotic cells that work together to modify, package, and transport lipids and proteins
endoplasmic reticulum (ER) a series of interconnected membranous structures within eukaryotic cells that collectively modify proteins and synthesise lipids
effector cell, tissue, organ or system that can cause a change in the value of a monitored parameter
enzyme substance (protein) produced by a living organism which acts as a catalyst to bring about a specific biochemical reaction
epithelial cells arranged in one or more layers to form a membrane that covers or lines body parts
eukaryotes multicellular organisms that are larger and more complex than prokaryotes
Golgi apparatus a eukaryotic organelle made up of a series of stacked membranes that sorts, tags, and packages lipids and proteins for distribution
homeostasis any self-regulating process by which biological systems tend to maintain stability while adjusting to conditions that are optimal for survival
lysosome membrane-bound cellular organelle originating from the Golgi apparatus and containing digestive enzymes
macromolecules large biomolecules like protein, fatty acids, carbohydrates and nucleic acids
macrophage a type of white blood cell that ingests foreign material
mitochondrion one of the cellular organelles bound by a double lipid bilayer that function primarily in the production of cellular energy (ATP)
negative feedback homeostatic mechanism that tends to stabilise an upset in the body’s physiological condition by preventing an excessive response to a stimulus
neuron specialised cell transmitting nerve impulses
normal range (with reference to homeostasis) range of values around the set point that do not cause a reaction by the control centre
nucleic acids the building blocks of DNA are nucleic acids. Nucleic acids are complex organic substances.
nuclear envelope the double-membrane structure that constitutes the outermost portion of the nucleus
nucleolus the darkly staining body within the nucleus that is responsible for assembling ribosomal subunits
nucleus cell’s central organelle; contains the cell’s DNA
organelle means “small organ” to denote membrane bound structures found floating in the cytoplasm of a cell
pH a measure of how acidic a solution is
phospholipid lipid molecule(s) attached to a phosphate molecule (one phosphorous combined with four oxygen atoms)
plasma membrane a phospholipid bilayer with embedded (integral) or attached (peripheral) proteins that separates the internal contents of the cell from its surrounding environment
positive feedback mechanism that intensifies a change in the body’s physiological condition in response to a stimulus
prokaryote single celled organism lacking a true membrane-bound nucleus, for example, bacteria
response the action that the effector takes as a result of the message from the control centre of a feedback loop
rough endoplasmic reticulum (RER) the region of the endoplasmic reticulum that is studded with ribosomes and engages in protein modification
ribosome a cellular organelle that carries out protein synthesis
RNA nucleic acid similar to DNA but containing ribose as the sugar molecule rather than deoxyribose
selectively permeable or semipermeable the characteristic of a membrane that allows some substances through but not others
set point ideal value for a physiological parameter; the level or small range within which a physiological parameter such as blood pressure is stable and optimally healthful, that is, within its parameters of homeostasis
sensor (or receptor) reports a monitored physiological value to the control centre
smooth endoplasmic reticulum (SER) the region of the endoplasmic reticulum that has few or no ribosomes on its cytoplasmic surface and synthesises carbohydrates, lipids, and steroid hormones; detoxifies chemicals like pesticides, preservatives, medications, and environmental pollutants, and stores calcium ions
tissue a group of connected cells in an animal or plant that are similar to each other and have the same purpose
vesicle a small, membrane-bound sac that functions in cellular storage and transport; its membrane is capable of fusing with the plasma membrane and the membranes of the endoplasmic reticulum and Golgi apparatus
vacuole a membrane-bound sac, somewhat larger than a vesicle, that functions in cellular storage and transport
Review questions
Chapter attribution
Content adapted from:
Anatomy and Physiology 2e (2022), by J. Gordon Betts, Kelly A. Young, James A. Wise, Eddie Johnson, Brandon Poe, Dean H. Kruse, Oksana Korol, Jody E. Johnson, Mark Womble and Peter DeSaix, is published by OpenStax https://openstax.org/details/books/anatomy-and-physiology-2e, and used under a CC BY licence.
Concepts of Biology (2013), by Samantha Fowler, Rebecca Roush and James Wise, is published by OpenStax https://openstax.org/details/books/concepts-biology, and used under a CC BY licence.
Microbiology (2016), by Nina Parker, Mark Schneegurt, Anh-Hue Thi Tu, Philip Lister and Brian M. Forster, is published by OpenStax https://openstax.org/details/books/microbiology, and used under a CC BY licence.
Media Attributions
- Figure 2.1 Homeostatic_Medicine © Songlin Wang & Lizheng Qin is licensed under a CC BY (Attribution) license
- Figure 2.2 Levels of organisation © J. Gordon Betts, Kelly A. Young, James A. Wise, Eddie Johnson, Brandon Poe, Dean H. Kruse, Oksana Korol, Jody E. Johnson, Mark Womble & Peter DeSaix is licensed under a CC BY (Attribution) license
- Figure 2.3 Simplified diagram of an animal cell. © domdomegg is licensed under a CC BY (Attribution) license
- Figure 2.4 Robert Hooke Microscope and cork © Robert Hooke adapted by Alejandro Porto is licensed under a Public Domain license
- Figure 2.5a Compound light microscope © Vicki Dunk is licensed under a CC BY (Attribution) license
- Figure 2.5b Dissecting microscope © Available under a Pixabay licence.
- Figure 2.6 Salmonella bacteria © (a) modification of work by CDC/Armed Forces Institute of Pathology, Charles N. Farmer, Rocky Mountain Laboratories (b) modification of work by NIAID, NIH; scale-bar data from Matt Russell is licensed under a Public Domain license
- Figure 2.7 Abnormal epithelial cells from the cervix © Ed Uthman is licensed under a CC BY-SA (Attribution ShareAlike) license
- Figure 2.8 Generalised structure of a prokaryote cell © Clker-Free-Vector-Images, available under a Pixabay licence.
- Figure 2.9 Generalised animal cell © Bingbongboing is licensed under a CC BY-SA (Attribution ShareAlike) license
- Figure 2.10 Phospholipid membrane with embedded proteins © OpenStax is licensed under a CC BY (Attribution) license
- Figure 2.11 Microfilaments, intermediate filaments, and microtubules compose a cell’s cytoskeleton © OpenStax is licensed under a CC BY (Attribution) license
- Figure 2.12 Nucleus and nuclear envelope © CNX OpenStax is licensed under a CC BY (Attribution) license
- Figure 2.13 Golgi apparatus © Openstax is licensed under a CC BY (Attribution) license
- Figure 2.14 Macrophage © Samantha Fowler, Rebecca Roush & James Wise is licensed under a CC BY (Attribution) license
- Figure 2.15 Golgi apparatus and endomembrane system © J. Gordon Betts, Kelly A. Young, James A. Wise, Eddie Johnson, Brandon Poe, Dean H. Kruse, Oksana Korol, Jody E. Johnson, Mark Womble & Peter DeSaix is licensed under a CC BY (Attribution) license
- Figure 2.17 0315_Mitochondrion_new © J. Gordon Betts, Kelly A. Young, James A. Wise, Eddie Johnson, Brandon Poe, Dean H. Kruse, Oksana Korol, Jody E. Johnson, Mark Womble & Peter DeSaix is licensed under a CC BY (Attribution) license
- Figure 2.17a Skeletal muscle cells © Berkshire Community College Bioscience Image Library is licensed under a Public Domain license
- 2.17b Ciliated epithelial tissue © Spike Walker. Wellcome Images is licensed under a CC BY-NC-ND (Attribution NonCommercial NoDerivatives) license
- Figure 2.17c Cerebellar nervous tissue © Berkshire Community College Bioscience Image Library is licensed under a Public Domain license
- 800px-Connective_Tissue_Hyaline_Cartilage_(26989336477) © Berkshire Community College Bioscience Image Library is licensed under a CC0 (Creative Commons Zero) license
- Figure 2.18 Polar bear © Ansgar Walk is licensed under a CC BY (Attribution) license
- Figure 2.19 Negative feedback loop adapted by Vicki Dunk is licensed under a CC BY-SA (Attribution ShareAlike) license
- Figure 2.20 Temperature negative feedback example © Vicki Dunk is licensed under a CC BY (Attribution) license
- Figure 2.21 Child birth Positive feedback loop © Openstax is licensed under a CC BY (Attribution) license